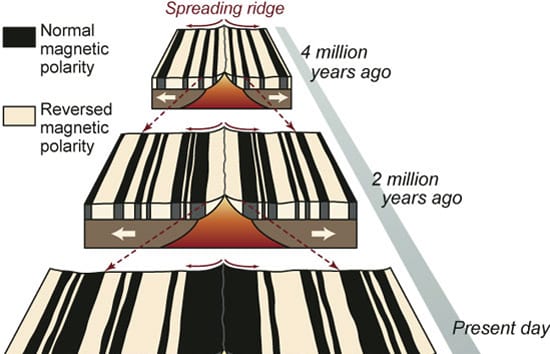
Paving the SeafloorBrick by Brick
New vehicles and magnetic techniques reveal details of seafloor lava flows
Most of Earth’s crust is manufactured at the bottom of the sea. Deep beneath the waves and beyond our view, magma erupts along a 40,000-mile volcanic mountain chain that bisects the ocean floors and encircles the globe. The lava flowing from these mid-ocean ridges solidifies into new ocean crust that spreads out and paves the surface of our planet.
That’s the “big picture.” But our ability to understand all the subtle and complex details of this fundamental, planet-shaping process is blocked by the oceans themselves. Miles of water prevent us from seeing the seafloor directly, and we can’t survive the darkness and high pressures at the seafloor to explore it firsthand.
Exploring from afar
Imagine explorers from another planet hovering in a spaceship high above a large American city. From this lofty perspective, the explorers would deduce that the urban landscape was not natural, but constructed somehow in particular ways.
To understand this landscape, they would want to know: When were individual structures made? Of what materials? In what sequence? How are the roads, buildings, and sidewalks erected? Why are they located where they are? Which came first? The extraterrestrials would have to zoom down to get a closer look and perhaps take some close-up photos and samples to untangle the myriad factors that cumulatively result in cities.
For ocean scientists to zoom down and take a closer look at the seafloor requires specialized equipment. In the past decade, we have taken big technological leaps. New undersea vehicles have given us unprecedented access to the seafloor and new abilities to collect previously unattainable data.
Our goal is to understand the seafloor processes that turn molten lava into fresh crust. In particular, we are developing an innovative technique, measuring the magnetic properties of very young seafloor rocks. It is revealing new details of how, where, and when lava erupts, and how it flows and accumulates on the ocean floor.
Telltale magnetic clues
As hot lava erupts, magnetic iron oxide crystals within it (magnetite) orient themselves to align with Earth’s magnetic field. When the lava cools and solidifies, its magnetic crystals are “flash frozen”—pointing “north.” The rocks’ magnetic direction, or “polarity,” is preserved.
But the magnetic north pole has not always been where it is now. Throughout Earth’s 4.6-billion-year history, its magnetic field has flip-flopped several times—with the magnetic north sometimes facing south, or vice versa as it is today.
The time periods when many of these magnetic reversals occurred are well documented. So seafloor lavas provide a built-in chronometer or calendar that we can use to determine when they were created. Thus, the continually forming ocean crust is kind of a tape recorder of Earth’s magnetic field history.
Magnetic ‘zebra stripes’
In the late 1960s, this phenomenon provided crucial evidence confirming that the seafloor was indeed spreading apart. This concept is at the core of the revolutionary theory of “plate tectonics.”
In the early 1960s, scientists analyzed the magnetic properties of rocks in terrestrial outcrops and developed a timescale that chronicled the reversals of Earth’s magnetic field. At sea, scientists collected magnetic data from sensors towed from ships and found remarkable magnetic patterns in seafloor rocks that were subsequently correlated with the magnetic reversal “clock” established in terrestrial rocks.
On both sides of mid-ocean ridges, scientists found a pattern of alternating magnetic “zebra stripes.” “Black” stripes represented rocks imprinted when Earth’s magnetic field was in a normal direction, as it is today, and “white” represents rocks imprinted when the field was in a reversed direction.
The stripes ran parallel to the ridges, and the pattern was astonishingly symmetrical on either side of the ridges. This mirror image could form only if new seafloor was created at the crests of ridges (with the prevailing magnetic signature) and then spread outward in both directions.
A new way to measure ‘young’ rock
Historically, magnetic data were collected with magnetometers towed at the ocean surface by research ships. They provided a large-scale picture (like the view of a city from a high-flying spaceship) of how ocean crust forms over many millions of years and hundreds of miles.
To get a more detailed view, we began to look at rocks less than 100,000 years old and within just a few miles of the ridge crest. This was problematic, because the most recent magnetic reversal occurred 780,000 years ago. We had no way of finding the age of lava younger than this.
To solve this dilemma, we began to examine not only the polarity of Earth’s magnetic field, but also the strength of the field, or intensity. The intensity of Earth’s magnetic field has also varied dramatically through time, and ocean floor sediments have preserved a record of Earth’s magnetic field intensity over the past few hundred thousand years.
So while magnetic polarity measurements give us ages within millions to hundreds of thousands of years, intensity measurements give us ages within thousands to tens of thousands of years—a minute hand on the magnetic clock. Thus, within a patch of young lava with the same magnetic orientation, we can distinguish older and younger rocks, and we can begin to unravel the sequences in which they were deposited on the seafloor.
Unprecedented seafloor access
To detect magnetic intensities, we must take measurements within meters of the rocks—something we just could not do until recently. In 1993, we received an opportunity to use a magnetometer aboard the submersible Alvin, and we confirmed for the first time that we could detect strong magnetic intensity signals in freshly erupted lava. The invention and development at WHOI of the Autonomous Benthic Explorer (ABE) in the mid-1990s made it possible to measure magnetic intensities over wide swaths of seafloor lava.
ABE can maintain a stable ride and a constant altitude over changing seafloor contours—features that make it well suited for collecting high-resolution magnetic measurements. Over several hours, it can survey 20 to 25 kilometers of seafloor. At the same time, its sonar can take measurements to create fine-scaled topographical maps.
The maps give us detailed, three-dimensional pictures of contorted amalgamations of lava flows. By superimposing magnetic measurements on these maps, we can distinguish the extent and volume of individual lava flows in the upper crust and tell when they erupted. We can begin to unravel how flows are buried by subsequent flows like a deck of cards. Alvin plays a crucial complementary role, giving us an essential visual picture of the seascape and the ability to sample fresh lava for precision dating.
Many bursts or a ‘crack of doom’?
My colleagues in this National Science Foundation-sponsored research—Hans Schouten, Dan Fornari, and Ken Sims at WHOI, and Jeffrey Gee at Scripps Institution of Oceanography—focus on the ocean bottom because seafloor lava flows are young and not yet buried by thick muds. They also have not lost their magnetic intensity due to chemical alteration with seawater, so they accurately record Earth’s recent magnetic field history.
The lavas found at mid-ocean ridges erupt more often, are better preserved, and are less disturbed than those found on continents. These attributes give us an opportunity to get a more “brick-by-brick” understanding of how the surface of Earth is paved.
For example, does lava erupt at a central “crack of doom” atop a ridge, and then spill over and cascade downhill to bury older lava flows (as portrayed in the right side of the top diagram)? Does it burst from several outlying magma chambers through narrow channels to create discrete patches of seafloor (as in the left side of the top diagram)? Or are both processes at work?
Answers to these questions will delineate the width of the zone along mid-ocean ridges in which new seafloor crust is formed. It will also reveal how frequently and periodically ridge eruptions take place—which, in turn, will tell us about the heartbeat of magma movements deeper in the earth.
Slideshow
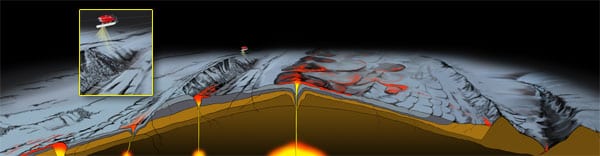
Slideshow
- How is the seafloor paved with lava?
The development of ABE, the Autonomous Benthic Explorer, made it possible to fly close enough to the seafloor to measure magnetic intensities of young seafloor lava. Such measurements will give scientists the ability to unravel the convoluted processes by which lava carpets the seafloor. Scientists are investigating whether fresh lava (red) erupts from a central point in the mid-ocean ridge (as depicted above) and cascades downhill to overlay older lava flows (gray), or whether lava erupts from several isolated, outlying magma chambers to create discrete patches of seafloor. Or perhaps both processes occur. - By superimposing magnetic measurements on detailed seafloor topography maps like this one, scientists can distinguish how, when, and where individual lava flows occurred. Younger lava has the highest magnetic intensities (red and yellows). Above, the most recent lava flow erupted from the ridge axis, overlaid older lava flows, and pooled to the left of the axis.
- Magnetic ‘zebra stripes’
Seafloor lavas have built-in magnetic “clocks” that reveal their age. When seafloor lava solidifies at the seafloor, its magnetic crystals are quenched in alignment with Earth’s magnetic field, and the rocks’ magnetic “polarity” is preserved. But Earth’s magnetic field has reversed many times over the planet’s history—with the magnetic north sometimes facing south, or vice versa, as it is today.
New seafloor is created at mid-ocean ridges (with the prevailing magnetic signature) and spreads out in both directions, creating a symmetrical “zebra-stripe” pattern of alternating rocks with either “reversed” or “normal” polarity.