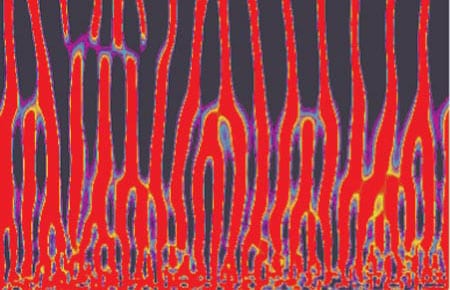
Melt Extraction from the Mantle Beneath Mid-Ocean Ridges
As the oceanic plates move apart at mid-ocean ridges, rocks from Earth’s mantle, far below, rise to fill the void, mostly via slow plastic flow.
Spring 1998 — As the oceanic plates move apart at mid-ocean ridges, rocks from Earth’s mantle, far below, rise to fill the void, mostly via slow plastic flow. As the rock approaches the top of its journey, however, partial melting occurs, so that the upper 6 kilometers of oceanic crust are composed of melts, which both erupt on the seafloor as lava and crystallize beneath the surface to form what are known as “plutonic” rocks.
Melt forms in tiny “pores” along the boundaries between different crystals, and the pores form an interconnected network of tubes 1 to 10 microns in diameter. Since the melts are less dense than the residual solids, they are relatively buoyant and move upward faster than the rest of the upwelling mantle. The exact process by which very small amounts of melt rise to form voluminous lava flows is the subject of some controversy. We do know that:
1) melts must rise through the upper 30 kilometers of the mantle without equilibrating with the surrounding rocks, and
2) melt forms in a region several hundred kilometers wide and then coalesces into a narrow band just a few kilometers wide—the mid-ocean ridge—where igneous crust is formed by crystallization of magma.
Studying processes that take place within the earth is not easy, but plate motions occasionally thrust pieces of crust to the surface that provide windows to the interior: Outcrops of Earth’s lower crust and upper mantle are exposed in ophiolites, slices of oceanic plates that are found on land. They generally occur in mountain belts along continental margins and are usually tilted, so that in the largest ophiolites the erosional surface exposes rocks that ordinarily reside 10 or even 20 kilometers below the seafloor. We turned to ophiolites to address the question of how slow, porous flow preserves disequilibrium between melts and the upper 30 kilometers of residual mantle rocks.
The process by which ophiolites are thrust onto the continental margins is uncertain. This raises a basic issue: The rocks in ophiolites are certainly formed at submarine spreading centers similar to the mid-ocean ridges, but they may be exceptional in their composition or structure—after all, “normal” oceanic crust is under water! Furthermore, like all exposures of ancient rocks, ophiolites only preserve an indistinct record of their formation. For questions regarding how representative they are, how hot they were, how fast they formed, scientists will always look to direct observations of active mid-ocean ridges.
However, rocks in ophiolites closely resemble the rocks sampled by dredging and drilling in the deep ocean basins, and the vertical structure of ophiolites corresponds well to the structure of oceanic plates inferred from geophysical measurements. Because they expose very deep rocks, and because their three-dimensional structure can be determined directly by geologic mapping, ophiolites provide a complete view of the oceanic plates’ internal geometry that is unmatched by any sampling or imaging technique used at sea. For example, the deepest holes so far drilled into submarine oceanic plates are 1.5 to 2.5 kilometers deep, about 10 times shallower than the deepest exposures in large ophiolites. And drill core is only 6 centimeters in diameter, whereas deep exposures in ophiolites extend for hundreds of thousands of square kilometers.
Because of these factors, there is an ongoing dialogue between scientists conducting seagoing research and those involved in ophiolite investigations that is essential to our understanding of the structure and genesis of oceanic plates.
Several types of melt extraction feature can be observed in the mantle section of an ophiolite located in Oman. They range from “dikes,” fractures filled with minerals crystallized from a melt, to “replacive dunites,” rocks composed only of the mineral olivine. Both of these types of feature are hosted within the normal mantle rocks, called peridotites, that are composed mainly of the minerals olivine and pyroxene. Our work focuses on the replacive dunites, which are formed when melt migrating by porous flow, saturated only in olivine, dissolves all the pyroxene from the rock. Dunites in the Oman mantle section show a deformation pattern that is sub-parallel to the paleo-seafloor, like the surrounding peridotites, indicating that the dunites must have formed in the region of mantle upwelling beneath the spreading ridge.
Our data show that replacive dunites in Oman formed within and around conduits of focused porous flow of melt deep in the mantle, and that the dunites were in equilibrium with the melts that formed the crust. Focused porous flow through dunites satisfies the constraint that melt must be transported through the upper 30 kilometers of the mantle without equilibrating with residual perido-tites. The width of the largest dunites exceeds 50 meters. Melts moving by porous flow in the interiors of such large dunites are separated from residual peridotite by many meters of dunite and thus escape chemical interaction with residual peridotite even though the melts are moving slowly.
How do dunites form? One possibility is that they simply arise as a result of reaction during porous flow. In the late 1980s, a group of hydrologists at the University of Indiana, led by Peter Ortoleva, conducted theoretical studies of porous flow of a solvent through partially soluble rock. They found that the coupling of flow and dissolution leads to the formation of high porosity channels that focus porous flow. Small perturbations in the initial porosity will grow exponentially because of a positive feedback loop: More fluid flow passes through areas with higher porosity, increasing the local dissolution rate, which increases the porosity, which in turn leads to yet higher flow velocities.
Ortoleva’s group and several other labs developed a “solution front” theory, in which undersaturated liquid comes into contact with the soluble porous medium along a planar front perpendicular to the liquid flow. MIT/WHOI Joint Program graduate student Einat Aharonov used sophisticated mathematics and new modeling techniques to show that even in a system where the liquid and solids maintain equilibrium everywhere, if the solubility of the solid in the liquid increases downstream, the result will be the exponential growth of dissolution channels.
Aharonov’s analysis also predicted the spacing between the fastest growing channels in the initial stages of channel growth. This spacing is proportional to the average flow velocity. As channels grow and become more permeable, the flow velocity increases. Based on this result, we predicted that over long time periods and long distances, natural systems would also produce networks with decreasing numbers of channels as a function of increasing time, and as a function of distance downstream. Such a channel network, coalescing downstream, is schematically illustrated in Figure 1. We called this picture Einat’s Castle.
Aharonov’s calculations and simulations were focused on the initial, infinitesimal stages of channel growth. More recently, Marc Spiegelman (Lamont-Doherty Earth Observatory, Columbia University) has modeled the process continuing to finite times. Many features that are transient in the initial stages reach steady state at longer times. Most importantly, the number of channels vs. distance downstream becomes steady, producing a coalescing drainage network (Figure 2) that is remarkably similar to the simple prediction in Einat’s Castle.
As for many coalescing channel systems in nature, the networks in Figures 1 and 2 show a “power law” relationship between the number of channels and the flux (volume transported per unit time) in the channels (Figures 3A & B). This arises because the flux doubles when two channels meet to form a larger one downstream, and this doubling is multiplied at each junction. This explains the slope in Figure 3A for Einat’s castle.
It is interesting to note that Theodore Wilson (University of Minnesota) in 1976 found the same kind of relationship, with conservation of flux downstream in human bronchial tubes. Their geometry is governed by an additional constraint that the cross-sectional area of the tubes is conserved at junctions. Such a branching geometry was first proposed by Leonardo da Vinci to account for the branching properties of some kinds of trees. The combination of downstream conservation of flux and cross-sectional area produces a power law relationship between the number and radius of the tubes in such a branching system (Figure 3C).
None of this “proves” that the dunites we observed in the Oman ophiolite formed solely as a result of dissolution channeling. In addition to dissolution channeling, dunite formation may also have been influenced by other processes including focusing of melt in zones of localized deformation, “suction” of melt into the region of plate separation beneath ridges, focusing of melt flow beneath permeability barriers, especially near the top of the upwelling mantle column, and perhaps the formation of dunite in porous reaction zones around melt-filled cracks. All of these processes may operate—in different times and places—and it is not yet clear which are dominant in melt extraction from the mantle beneath oceanic spreading ridges.
It was with the hope of providing vital new data to discriminate between these possibilities that we conceived the idea of taking photographs from a blimp. We reasoned that the preferred channel spacings and coalescing networks that arise in simple models of porous flow through a soluble solid matrix might be found in the mantle dunites of the Washington Cascades. If a regular spatial distribution of dunites existed and could be photographed by a blimp-mounted camera, it might indicate an important role for dissolution channeling during dunite formation.
We were inspired by detailed, photomosaic maps of RMS Titanic made by the remotely operated vehicles Jason and Argo, and reasoned that we should be able to produce similar maps on land. In 1996, WHOI Associate Scientist Greg Hirth and I received a WHOI Mellon Independent Study Award that allowed us to purchase an 8-meter-long blimp, 3 meters in diameter, from AdverBlimp, in Sioux Falls, South Dakota. It is similar to blimps that fly over shopping malls and car dealerships, announcing a sale or the opening of a new showroom. BOLO (Blimp for On Land Oceanography) can lift about 20 kilograms. We used a helicopter to fly helium into a backcountry campsite, inflated the blimp, hung a camera beneath it, and photographed rock outcrops in alpine meadows at about 2,000 meters elevation.
In geology it is impossible to escape the influence of history. Thus, we did not expect to reveal a simple, coalescing network. The mantle that will undergo melting as it rises beneath a spreading ridge has a complicated prior history that began with accretion of material that formed Earth, and has continued through many episodes of crustal formation and recycling. This gives rise to compositional variations, which in turn produce varying amounts of melt. In addition, the crystals that form the mantle have been stretched and aligned, “lineated,” by billions of years of convection. Because of these processes, melt flow networks encounter pre-existing structures that guide their geometry, making predictions of their patterns difficult. The inescapable effects of history, potentially unique for each specific region, constitute a major barrier to theoretical prediction in the earth sciences. A more useful application of a model such as ours is to try to gain insight into general principles governing coalescing networks of porous channels. One such principle is a tendency toward exponentially increasing flux with an exponentially decreasing number of downstream channels (Figure 3).
Figure 4 illustrates one photo frame taken from the blimp along with a combination of about 50 such frames into a digital photomosaic. Creation of this mosaic was greatly aided by the fact that the outcrops are almost perfectly flat! We made all our photographs with the camera lens oriented perpendicular to the plane of the outcrop, from a constant distance away. Furthermore, we were lucky in that all of the channels in the dunites of this area are oriented perpendicular to the plane of the outcrop, which greatly eases determination of their true size in three dimensions.
In Figure 4, the channels are in black, residual mantle peridotite is grey, and areas covered by soil or vegetation are white. Having created this image, our next step was to measure the size and number of dunites. We did this by adding a grid of straight lines, aligned perpendicular to the general trend of the dunites. We asked our computer to find all the intersections between the lines and the dunites, and measure their length. Then we combined and analyzed the measurements in terms of the number of intersections with a given length. These data are shown in Figure 5 for several outcrops.
It is striking that the width of the dunites (intersection length) has a power law relationship to their number, with a negative slope, as in the size/frequency plot for human bronchial tubes in Figure 3C. The presence of such a regular structure suggests that all the dunites were active at once, and formed a coalescing network in which small conduits of focused flow within many small dunites were connected to a few large conduits within large dunites. The constancy of the slope from outcrop to outcrop gives us confidence that the measurement is “robust”—not an artifact due to a limited number of observations.
Human bronchial tubes are open cylinders, whereas conduits within dunites in the mantle are channels of high porosity. Thus, the slopes in Figures 3C and 5 should not be the same. We can reproduce the observed slope for the dunites in Figure 5 with a simple scaling relationship in which flux is conserved, channels join in pairs with similar sizes, and the porosity varies from 5 percent in the biggest dunites to 0.5 percent in the smallest ones.
Much more data analysis will be required in order to adequately test the theory that a chemical dissolution process is responsible for this structure. However, the linear relationship in Figure 5 is exciting for many reasons. For melt extraction, it suggests that the relationship between width and number of channels might be generally predictable. If so, we can extrapolate this relationship to a much larger area, such as the cross-sectional area of the mantle beneath an active mid-ocean spreading ridge, as schematically illustrated in Figure 6. This approach could be valuable because we will never be able to observe such a huge area directly. We can then ask, are there dunite channels wide enough and numerous enough to account for chemically isolated transport of melt from deep in the melting region to the base of the crust? Our provisional answer is “yes.”
Looking beyond the specialized field of melt extraction, our results on the mechanisms and consequences of flow focusing become even more exciting. In 1976, Wilson emphasized the fundamentally important point that the coalescing network of bronchial tubes in the human lung is optimally efficient. For a system with a single large inlet (your throat), and the requirement to disperse air to the surface of thousands of capillaries less than a millimeter in diameter, the geometry of Figure 3C minimizes energy loss due to friction of moving air along the tube walls. In terms of thermodynamics, the bronchial network minimizes entropy (the amount of energy unavailable for useful work in a system undergoing change).
Perhaps it is not surprising that human lungs are optimally efficient. After all, organisms on Earth have had billions years of trial and error to work out the bugs in their design. However, some chemical systems develop similar structures in minutes. Nobel-prize-winning chemist Ilya Prigogine (University of Texas at Austin and International Solvay Institutes, Belgium) showed that, on laboratory time scales, some systems, which are open to chemical transport and which remain close to equilibrium, tend to find a steady state that minimizes entropy production. Again, perhaps this is understandable since chemical organization is the statistical result of countless molecular interactions, each of which happens in nanoseconds.
It may be that a variety of Earth systems—porous channels, mantle convection, rivers, faults—all tend to develop geometries that minimize entropy production. They might not always reach an optimal structure, given the constraints of geologic history. However, just understanding this tendency could lead to the development of valuable predictive tools, and provide a unifying factor in the study of disparate natural phenomena.
Initial research described in this article was supported by the National Science Foundation. Purchase and use of BOLO (Blimp for On-Land Oceanography) in the Washington Cascades was supported by a WHOI Mellon Independent Study Award.
Peter Kelemen has been at WHOI, as a postdoc and then a scientist, for 10 years. During this time he and his wife, Rachel Cox, have acquired a house, two kids, and Rachel’s Ph.D. in physiology from Boston University Medical School. During the initial four of his six years as an undergraduate, Peter was an English and philosophy major at Dartmouth College. He then realized he would need to get a job when he graduated. In the meantime, he had learned technical climbing techniques. He reasoned that it would be best to work outside in the mountains, and so switched to a major in earth sciences. In 1980, Peter and some friends founded Dihedral Exploration, a consulting company specializing in “extreme terrain mineral exploration.” From 1980 to 1991, he split his time between geological research and mineral exploration, in the process obtaining a Ph.D. from the University of Washington. As a mineral exploration consultant and research scientist, Peter has been fortunate to work in the mountains of California, Oregon, Washington, British Columbia, Peru, the Yukon, Alaska, the Indian Himalaya and Karakorum Ranges, East Greenland, and even along the Mid-Atlantic Ridge, as well as in the Oman ophiolite.
Slideshow
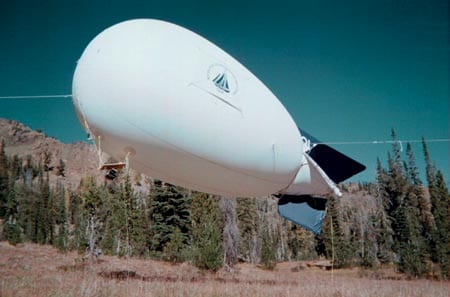
Slideshow
- Photographs for studies of a Washington Cascades ophiolite were taken from BOLO (Blimp for On Land Oceanography) tethered at a height of about seven meters
- Diagram of rising mantle, convection, and melt region.
- Red lines show known ophiolites, slices of oceanic crust that have been exposed on land by tectonic forces. Ophiolites allow scientists to study the three-dimensional structure of the oceanic crust and shallow mantle directly rather than by remote means beneath the seafloor. (After W. P. Irwin and R. G. Coleman, U.S. Geological Survey, 1974.)
- Figure 1. Schematic illustration of a porous dissolution channel network coalescing downstream, based on theoretical work of MIT/WHOI graduate student Einat Aharonov and others.
- Figure 2. Results of numerical modeling of the formation of porous dissolution channels in the mantle by Lamont-Doherty Earth Observatory scientist Marc Spiegelman and others.
- Figure 3. Plots illustrating the relationship between the number of channels and flux in coalescing flow networks. Plot A is based on the schematic network in Figure 1. Plot B illustrates results of numerical modeling with points used in fitting the curve marked in red. Plot C is based on the work of Theodore Wilson (University of Minnesota), who showed that coalescing human bronchial tubes conserve cross-sectional area as well as flux. This is the optimally efficient geometry for respiration.
- Figure 4. Maps made using BOLO, the Blimp for On Land Oceanography. The color illustration is an example of a single photograph of an area about 3 meters across, and the grayscale image is a map of an outcrop about 30 meters across, created from a mosaic of about 50 photographs. Note that, though the dunites channels appear small here, they are actually several meters wide.
- Figure 5. Plot showing results for all the dunites mapped in the Washington Cascades ophiolite in terms of the number of dunites with a particular width. As for coalescing channel networks, the dunites show a power law relationship with a negative slope between their width and their number. Points used in fitting the curve are shown in red. The blue lines illustrate that the observed slope can be explained as the result of a network of interconnected, porous dunite channels, with porosity varying from a maximum of 5 percent in the largest channels to a minimum of about 0.2 percent in the smallest ones.
- Figure 6. At left, a schematic cross section of the mantle section of the Oman ophiolite illustrates the relationships between residual mantle peridotites (gray), dunites (red), and dikes (black). Light lines show the trend of deformation features ("foliation") in peridotite, which are sub-parallel to the paleo-seafloor. At right, constraints from the mantle sections of ophiolites, and theoretical studies of reactive porous flow of melt through partially soluble rocks, can be used to infer that melt extraction beneath mid-ocean ridges occurs in a coalescing network of porous dunite channels.